Are Nature’s Laws Really Universal?
Are the laws of physics the same everywhere in the Universe and throughout its entire 14 billion year history? This basic question is the motivation for research I’ve been undertaking for more than 10 years with several colleagues.
Below is an explanation of this work in layman’s terms. Alternatively, Watch me talk about it. I also wrote an article for Australian Physics magazine about it. See the research news items and my publications for more detail.
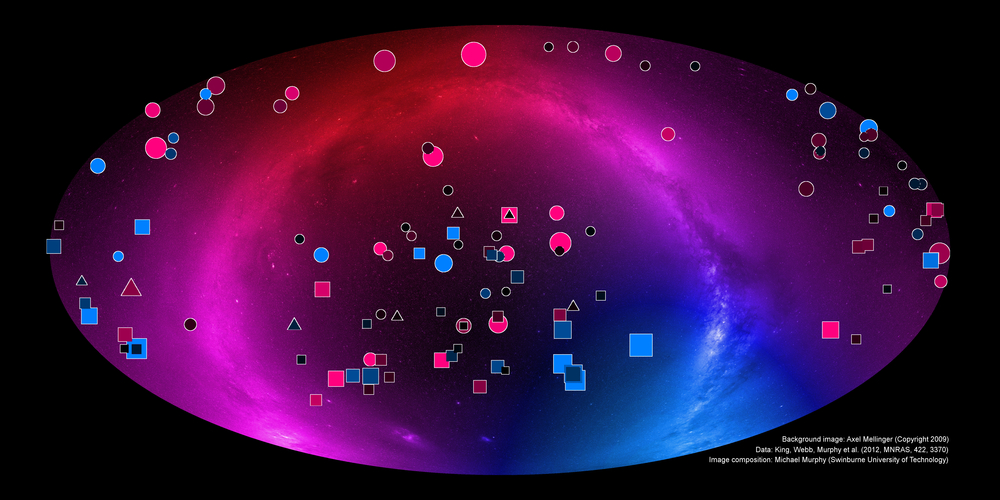
Do the laws of Nature vary across the sky and/or throughout the Universe? |
Quick links
Team
This is a collaborative effort involving mainly (recently-graduated PhD student) Dr. Julian King, Prof. John Webb and Prof. Victor Flambaum at UNSW (Sydney, Australia) and myself at Swinburne University of Technology (Melbourne, Australia). Other collaborators are Prof. Bob Carswell (Institute of Astronomy, University of Cambridge, UK) and Matthew Bainbridge (UNSW).
Basics
First, a little ‘FAQ’ about the basics about fundamental constants, particularly the fine-structure constant – the strength of electromagnetism – often called alpha (α):
What is a fundamental constant?: @ Wikipedia, @ NIST
What is the fine structure constant?: @ Wikipedia, @ NIST, @ Physlink
How are the constants measured?: @ Physics Today
Put briefly, a fundamental constant is a number that is central to a given theory – that is, to calculate/predict the results of an experiment, you need to know that number. But you can’t use known theories to calculate that number – it must be measured in an experiment. In essence, these numbers are fundamental because we have no idea where they come from! And since no-one knows how to calculate their values, and because we do find the same value with different experiments conducted at different times/places, we assume that these numbers are, in fact, constants. The experiments we conduct tend to be limited to laboratories on the Earth during the last 100 years or so. But what if the constants are/were different in different places in the universe or at different epochs in cosmic history? An experiment should test the constancy of the constants in these extreme cases.
Our experiment picks on one very well known constant: the fine structure constant, alpha (α). This constant is the central parameter in electromagnetism – the theory of how light and matter interact. Alpha is a combination of other constants that you might be more familiar with: α = e2/hc where c is the speed of light, e is the charge of an electron and h is Planck’s constant. Thus, alpha is important for a relativistic (i.e. c) quantum mechanical (i.e. h) theory of electromagnetism (i.e. e). But alpha is, in some sense, more fundamental than these other constants (see Aside – is c or e varying?). Alpha is known to exquisite precision from laboratory measurements:
Experiment
Our investigation is astrophysical in nature. We look back in time through most of the history of universe to compare the value of alpha back then with what we measure in the laboratory on Earth today. We also look in as many different directions through space as we can, just in case alpha varies from place to place in the universe as well (or instead). To do this we have used the largest optical telescopes in the world — The Keck I 10-m telescope on Mauna Kea, Hawaii and the ESO Very Large Telescope (VLT) in Chile — to record spectra of extremely distant quasars. A diagram of a quasar spectra illustrating our experiment is shown below.
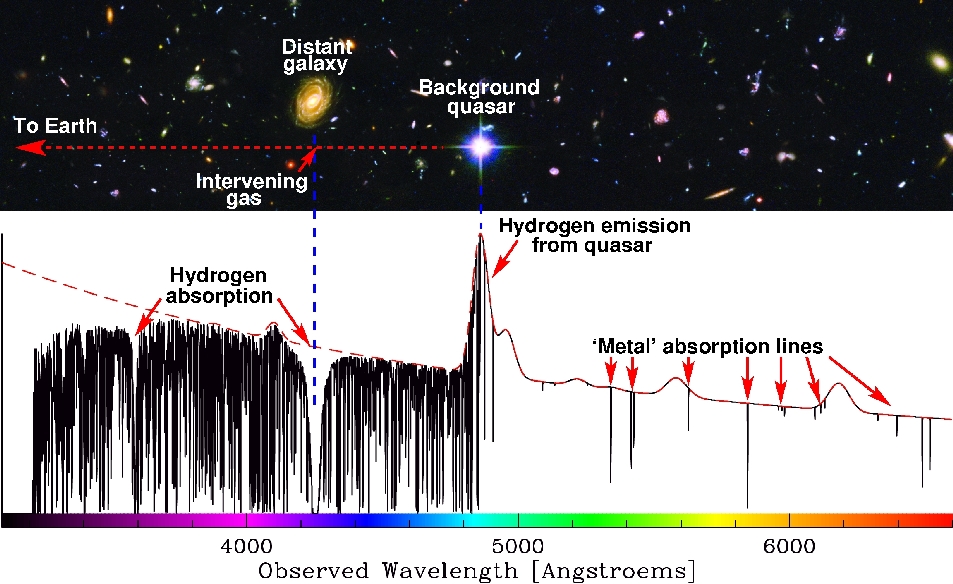
Figure 1: We look back from Earth to a distant quasar and spread the light out into a spectrum. As the light travels to Earth it traverses an intervening absorption cloud. The atoms in the cloud imprint the quasar spectrum with their “fingerprint” absorption lines (labelled as “‘Metal’ absorption lines”). We compare this fingerprint with that found in laboratories here on Earth to infer any possible changes in the fine-structure constant. Note: High-quality versions of this plot, with and without annotations, and variations of them, are provided here. |
Quasars are extremely massive (about a billion solar mass) black holes that rip apart and suck in any surrounding material. They reside at the centre of some galaxies and pull in the surrounding stars and gas. The inspiralling material heats up as it collides with other material causing the quasar to shine extremely brightly. In fact most quasars outshine their host galaxies which contain more than 100 billion stars. A massive black hole also resides at the centre of our own galaxy – The Milky Way – but it is not massive enough to become a quasar. Quasars are so bright and so compact that they can be seen even when the quasar is many billions of light years away (to compare, the age of the universe is about 14 billion years).
Quasars are very interesting in themselves but, in our experiment, we use the quasars only as background sources of light to reveal our real targets of interest, intervening foreground galaxies. These gas clouds in these galaxies are mostly made up of hydrogen (the most abundant element in the universe) but also contain ionized traces of heavier atoms such as magnesium, aluminium, silicon, chromium, iron, nickel and zinc — metallic atoms familiar to us here on Earth.
The metallic atoms absorb some of the quasar light on its 10 billion year journey to the Earth. This absorption is characteristic of each atom, much like a fingerprint is characteristic of each person. As we receive the light at the telescope, we spread it out into its colours – we form the spectrum of the quasar light – to reveal each atom’s fingerprint. See Fig. 1 above for a schematic explanation of this.
Previous results
We compare the atomic fingerprints in the quasar spectra with those measured in laboratory experiments here on Earth. Rather than finding them to be the same, as one might expect, on average we see very slight differences between the quasar and laboratory spectra: the lines making up the fingerprints appear at slightly different wavelengths/colours than expected! The separation between the absorption lines is controlled by the fine structure constant, alpha, and so we interpret these differences as changes in alpha.
Back in 1999 we published our first analysis of 30 distant absorption clouds observed with the Keck 10-m telescope in Hawaii. Those results revealed tentative evidence for a smaller alpha in the distant absorption clouds compared to the Earth’s value.
In 2001 we published stronger evidence for a smaller alpha in more, and even more distant, galaxies. Two independent samples of Keck spectra seemingly revealed the same effect. We also throroughly explored possible “systematic effects” – artefacts in the data or our analysis which might spuriously mimic a varying alpha – but we found none that could easily explain our results. A The New York Times article nicely summarizes the 2001 results.
In 2003 we published a very large third sample of galaxies, again from the Keck telescope and again showing evidence for a smaller alpha in the absorption clouds. By 2004 we had made 143 measurements of alpha – see Fig. 2 for a recent plot of our results – and, on average, all three independent samples all pointed towards a smaller alpha in the distant universe…
…at least in the general direction of the sky seen by the Keck telescope, Hawaii!
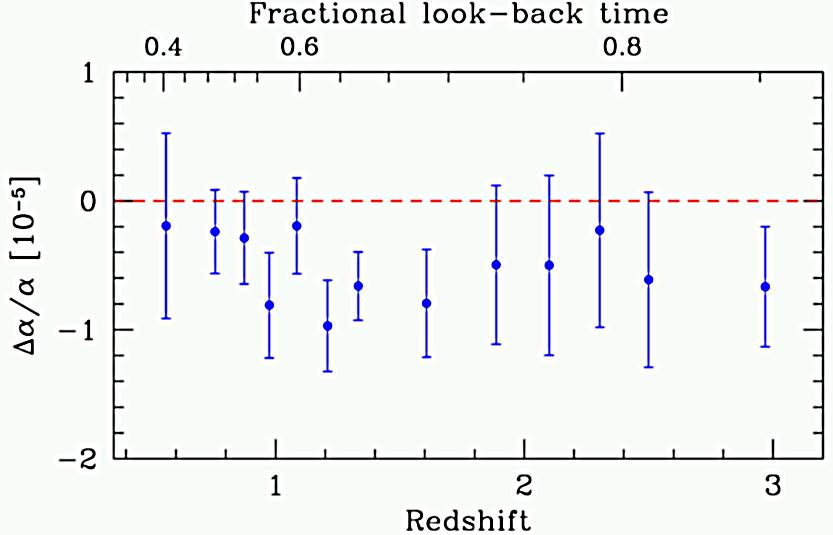
Figure 2: Our measured fractional change in the fine structure constant, alpha=e2/hc, from our previous Keck results is plotted as a function of fractional look-back time (0=present day, 1=beginning of time, about 13.7 billion years ago). The corresponding cosmological redshift is shown along the bottom axis. Each point represents the average value of about 10 absorption systems. There is now evidence that alpha has evolved one part in 100,000 over the last 10 billion (or so) years. Another possibility is that alpha is simply different in distant regions of the universe from what we measure on Earth. But what does the VLT say? |
New results
Enter our new results from the Very Large Telescope (VLT), Chile.
It’s absolutely essential to check the Keck results on another telescope to make sure no subtle problems with the telescope spuriously mimicked a varying alpha in our results. So, over the past few years, we’ve been busily measuring alpha from publically-available quasar spectra taken with the VLT.
And here’s the strange thing: we do find evidence for a different alpha in these VLT galaxies, only this time alpha is, on average, larger in those galaxies compared to here on Earth, not smaller like in the Keck results.
Being in the southern hemisphere, the VLT sees, on average, a different part of the sky than the Keck telescope. So we checked how alpha varied in our results as we looked in different directions on the sky. To our surprise a simple consistency emerged: the variation in alpha is well-described by a big “dipole” on the sky. That is, if you look in one direction in the universe (the pole), alpha is larger. Look in the other direction (the anti-pole) and alpha is smaller. Look somewhere between and alpha is, on average, somewhat closer to the value we see here on Earth.
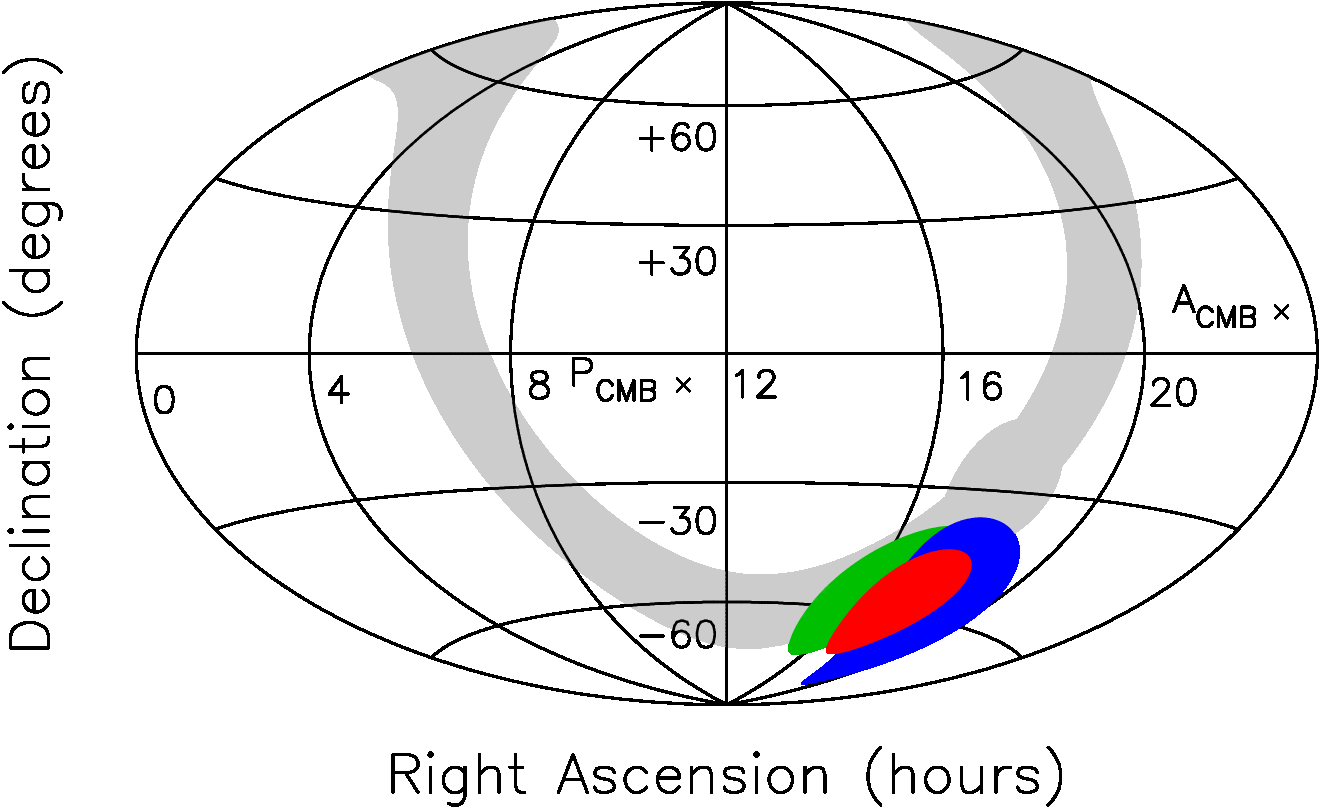
Figure 3: All-sky plot showing the dipole directions from the Keck (green), VLT (blue) and combined (red) samples. The “balloons” represent the 1-sigma uncertainty contours for the dipole directions. There’s about a 4% chance of the Keck and VLT dipole aligning as well (or better) than we see here by chance alone. |
How robust is this result? It’s always hard to tell exactly how reliable a result like this is, but, speaking strictly statistically, our data suggest the dipole signal we find only has a 0.004% chance of being a fluke. Scientists would say that the result is significant at the 99.996% confidence level. That sounds really robust! That’s why why we’ve reported the results. But extra special care is required when such surprising results like this emerge — extraordinary claims require extraordinary evidence!
One encouraging aspect of this result is that, if you treat the Keck and VLT results separately, the poles from each of them point in about the same direction on the sky. See Fig. 3 above. We calculate that there’s about a 4% chance of this being a fluke.
The pole of this “alpha dipole” is way down under. It lies in the little-known constellation of Ara (the alter), just to the south of the better-known contellation of Scorpius (the scorpion). The pole’s astronomical coordinates are roughly right-ascension 17 hours, declination -61 degrees.
Implications
Since alpha fixes the strength of the electromagnetic interaction, it plays an absolutely fundamental role in the working of atoms and how those atoms interact with light. In short, alpha controls most of the phenomena you see around you in everyday life. If the results above are confirmed by future experiments then does a change in alpha of 1 part in 100,000 over 10 billion years or from one side of the (visible) universe to the other mean anything for everyday life? There are several answers to this question.
The first and most naive answer is “No”. The change in alpha we observe means very little for everyday life because alpha won’t have changed by very much over the lifetime of humanity. This is not enough to cause atoms to fall to bits or for the Sun to burn its fuel at a terribly different rate.
But we should learn a lesson from history here. When Faraday discovered electricity in the 19th century, people often questioned him as to the significance of his discovery. At that time, Faraday could not possibly have imagined how society would become so completely dependent on electricity today. When Chancellor of the Exchequer, William Gladstone was invited to a demonstration of Faraday’s electrical equipment, Gladstone is reputed to have remarked “It is very interesting, Mr Faraday, but what practical worth is it?” Faraday is supposed to have replied “One day, sir, you may tax it.”. One doesn’t know at the present what everyday implications our discovery may have if it is shown to be correct – that’s one of the wonderful things about doing basic research like this!
More seriously, our results have the potential to revolutionise the way we understand the universe on all scales, from the subatomic to the universal. All of modern physical theory is based on the assumption that the laws of physics remain the same everwhere and everywhen. Physicists have what is called a “Standard Model” of the universe which allows them to explain all observed phenomena. This Standard Model cannot explain variations in the constants and so, if our results are correct, the Standard Model would need a complete overhaul: we will have discovered the first hint of a completely new set of physical laws, hitherto unseen and not to be understood for some time.
But perhaps we are closer to understanding varying constants than we think. Some modern theories which step outside the “Standard Model” have room for varying constants. In particular, theories such as string/M-theory allow for changes in the constants. These theories attempt to unify all the known forces of Nature (gravitation, electromagnetism, the strong and weak nuclear forces) by postulating the existence of extra dimensions in space. The extra dimensions (sometimes more than 20 in number!) are “compactified” or rolled up onto themselves so we don’t experience them in our usual (3+1)-dimensional universe. If the size of the extra dimensions changes (as do our three dimensions – ever heard the expanding universe?) then this could manifest itself as changes in the fundamental constants.
Future
Our results are by no means conclusive. We have thoroughly searched for other possible explanations for our results. Research science is constantly plagued by the problem of “systematic errors”. These errors mimic your result or somehow destroy it. They are notoriously hard to identify. And this is what we’ve been looking for in our results. But we still can’t find anything that explains our results besides a varying alpha! The new “alpha dipole” adds a new twist as well, making it even more difficult to understand how a systematic effect (or several) might have mimicked what we found.
The best approach in science is to always check (and re-check if necessary) your results using different equipment and analyses. We continue to analyse new, large datasets observed with the Very Large Telescope and Keck telescope. But, as mentioned above, there’s a famous saying in science: “Extraordinary claims require extraordinary evidence.”. Though we are claiming something quite extraordinary here, the evidence, though very strong, is not extraordinary enough. Yet. No one should really believe that constants are varying until another type of experiment confirms the results. Possibilities for other types of experiments include making very precise measurements of the fluctuations seen on the Cosmic Microwave Background sky — the radiation left over from the big bang. Another possibility is to measure very accurately the abundances of the elements that were produced in the big bang. But these methods have their own problems and systematic errors. But we’re hoping this will improve soon!
Probably the best chance of confirming our results is to search for varying alpha in the laboratory somehow, perhaps by comparing the ticking rates of ultra-precise atomic clocks. The current best precision is not quite high enough but atomic clock technology is improving extremely rapidly, so we may know for sure sooner rather than later.
Aside: Is it e or c varying?
Above I mentioned that alpha is made up of three other constants: α = e2/hc where c is the speed of light, e is the charge of an electron and h is Planck’s constant. Both laymen and scientists alike always ask whether we have any idea whether it’sc, e or h that varies. This frequently asked question has a subtle and often misunderstood answer. But it’s interesting, so read on!
In fact, one can never experimentally distinguish between a varying c or e because these quantities are always measured in some arbitrary units like meters, kilograms, seconds etc. Consider measuring the time it takes light to travel between you and me on Monday and then again on Tuesday. Imagine that the two answers were different. What does this tell you? You might conclude that the speed of light, c, has changed between Monday and Tuesday or, equally well, you could conclude that time has slowed/accelerated or that your measuring rods (i.e. meter rules) have changed length. These three conclusions are all equally valid and can not be distinguished by an experiment! But alpha is special because it is a dimensionless combination of other constants: alpha is just a number, i.e. no units! We can therefore measure changes in alpha unambiguously.
Some confusion has arisen recently in the literature about this question. The problem is that there exist well defined theories called “Varying Speed of Light” (VSL) and “Varying Electric Charge” (VEC) theories. For example, in VSL theories, it is indeed the speed of light that is considered to vary. But this is just a mathematical convenience: one could easily convert any VSL theory into a VEC theory! The only reason one chooses to label one particular theory a VSL or VEC theory is because that theory might look simpler (mathematically and intuitively) when considering a varying c or e. Essentially, the confusion is that the (arbitrary) names given to these theories mask their inherent duality (or triality if you include h!).